January 1962 Radio-Electronics
[Table of Contents]
Wax nostalgic about and learn from the history of early electronics.
See articles from Radio-Electronics,
published 1930-1988. All copyrights hereby acknowledged.
|
Two somewhat clichéd
sayings come to mind when reading this 1962 Radio-Electronics magazine
article on cathode emission research: "A picture is worth a thousand words," and
"settled science" is only a temporary thing. Author Dr. Aurelius Sandor was in
on the early (1930's) research of cathode rays, having been a (younger)
contemporary of Hans Geiger, of the eponymously named
counter fame. Basic
assumptions and practices applied by researchers for two decades inhibited
investigations into alternate means of generating, controlling, and displaying
fine detail of millimicron (1 millimicron ~ 3.937-8 inch) features.
The techniques presented here resulted in the type "outside the box" thinking
which often results in breakthroughs when many believed an immovable barrier had
been reached. Read on to see what I mean about the clichéd sayings.
Seeing is Believing in Cathode Research

The pattern on the tube face can be photographed together with other
instruments showing the time, pertinent voltages and other information, for a permanent
record.
By Dr. Aurelius Sandor*
Largest electron-emission microscope replaces abstract theory with visible reality
in search for better tube cathodes.
In the early days of the electron-optical science (in the beginning of the '30's)
I once respectfully called my professor, the famous H. Geiger of Geiger counter
fame, to watch a demonstration of an oxide cathode in my laboratory. He came, and
I pulled down the window shades with deep reverence. Out of the black nothingness
the brilliant landscape of the "moon" appeared on the rather small phosphor screen
of the cathode-ray tube, like a materializing ghost with craters, mares and criss-cross
channels, brightly scintillating in their versatile texture.
But the dynamic changes of the electron emission pattern suggested more than
just the topography of the moon, and the show took an even more dramatic turn when
- induced by an increase in cathode-heater voltage - the craters gradually covered
themselves with a dense veil and all sharp detail merged into a heavy, bright fog.
The relaxed voice of the otherwise nervous professor, colored with a shade of sentimentality,
was heard out of the dark: "Oh, my, Oh, my! Is this really possible?"
There was no doubt that Professor Geiger knew in all its implications the theory
that was hiding behind those mirages, but he could not suppress the sincere voice
of his relieved conscience, since somewhere, in a small corner of his great mind,
he had not been so unconditionally sure of the reality behind that theory.
To me, as a young engineer-physicist, this was quite an experience and I still
remember it with great respect every time a new pattern of similar astronomical
quality appears on our new emission microscope screen, a pattern greatly emphasized
by its present large size.

Fig. 1 - Schematic of the emission microscope tube.

Fig. 2 - The magnified images of cathode crystals are very sharp.
Fig. 3 - The odd case of decreasing emission with increasing temperature.
Fig. 4 - Vaporization of a portion of the cathode surface.
Fig. 5 - First stage in emission from tungsten dispenser cathode.
Fig. 6 - More uniform diffusion as barium comes to cathode surface.
Fig. 7 - The completely activated cathode.
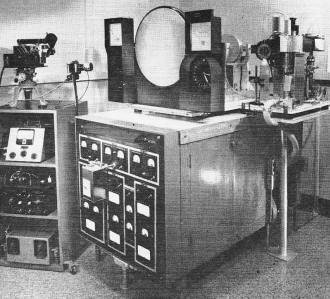
The complete electron microscope in the Bayside laboratory.
Electron Optics
The gadget used in the early demonstration was an early "electron emission microscope,"
a brother of the better known transmission electron microscope, but because of its
different physics unfortunately inherently limited in resolving power. The principles
of electron optics are abstract, but happily light optics offers analogies that
help us to explain them. Both electron optics and the optics that uses glass lenses
are based on the propagation of elementary masses which are related to electromagnetic
waves. In light optics, one speaks of "photon masses" of wave character; in electron
optics of "electron masses" also endowed with wave properties. The main difference
between the two is that electrons are electrically charged and photons are
not. So in electron optics specially shaped electric and magnetic fields have to
substitute for glass lenses; we find apertured anodes and in certain designs also
magnetic coils.
The emission microscope developed in our General Telephone & Electronics
Laboratories in Bayside, N. Y., represents a novel approach, conceived to conform
with modern research requirements. Complicated electric fields develop in the electron
gun, that also accommodates the exchangeable cathode to be viewed (Fig. 1). A first
and a larger second magnetic coil (lenses) surrounding the tube neck represent the
actual optical elements; this objective lens and this projection lens throw a true
and magnified electronic image of the 1/8-inch diameter cathode onto the full 21-inch
phosphor screen. The phosphor radiates visible light from the points of impact,
varying in shade according to the striking electron density.
Electron beams form images in the same way as light rays do. The focal length
of electron-optically shaped fields can also be changed simply by changing the field
strength value of the "lenses" (potentials, currents). Correction of lens defects
is of paramount importance, but corrections in electron optics are far more difficult
than in light optics. Hence the resolving power of 200 millimicrons obtained on
the screen with this design and amounting to only 80 millimicrons on a photographic
plate in a vacuum is a result not to be sneezed at, particularly when we consider
the extreme picture size. Photographers among our readers will appreciate this.
Magnifications on the screen may be varied from 7 to 300 times, followed, if so
required, by post-magnification with optical equipment.
The microscope tube shown in the schematic is a demountable type connected by
flexible vacuum bellows to the high-vacuum pump. More sensitive cathodes are investigated
in tubes that can be baked out thoroughly during evacuation before seal-off, to prevent
any chemical change on the cathode from occurring by reaction with gaseous residuals.
An automatic time drive located in the box on the instrument panel (see photo)
adjusts the cathode heating cycles; it takes hours to complete a series of investigations.
Time-lapse cinematography is also used to record and analyze sequences of cathode
development, until the desired electron activity is obtained, as can be judged from
the screen pattern.
The film strip also photographs the major experimental data, such as date, time,
frame number, pressure in the tube and the cathode temperature (which is derived
from amplified signals of an infrared sensor positioned outside the glass envelope,
opposite the glowing cathode).
If the pressure in the microscope tube rises accidentally above a permissible
value, visual and acoustic alarms go off and all electric power connections are
automatically cut. This is good practice, since the 15 kilovolts that are applied
could destroy delicate systems in case of electrical gas discharges, and possibly
initiate an implosion of dangerous dimensions.
Research on Cathodes
An electron tube is only as good as its cathode; its life starts and ends with
the electron source. The importance of this tube component justifies the elaborate
research in this field that started half a century ago with the alkaline earth oxide
cathode introduced by Wehnelt.
For some time, progress on thermionic emitters was slow because of the atomic
complexity of the physics involved. Although in the '30's electron optics had put
one foot in the door by making electron radiation directly visible on phosphor screens,
the subject was not followed up to its full extent - researchers did not realize
they had a potential tool for exploring the physics and technology of heated cathodes.
In those days - and even recently - they were aiming at too high magnification of
too small cathode areas. Therefore, acquainted with the non-optical cold field-emission
microscope that could reproduce the image of a point only, they underestimated the
early electron-optical microscope and ascribed limitations to it that did not really
exist.
In the General Telephone & Electronics Laboratories the line was successfully
picked up again. We worked on the philosophy that only the observation of simultaneous
local events over a large cathode surface could produce meaningful conclusions that
would be of value in promoting technical progress. This led to the design of the
large electron microscope on the cover.
Analyzing the brightness content (related to emission intensity) and the geometry
of the half-tone pattern on the luminous screen enables the cathode physicist to
draw realistic conclusions about the emission mechanism. In the past, projected
- and therefore speculative - conclusions were drawn about details of the active
cathode surface. Diode type current measurement was the tool employed; the actual
elements of emission could not be pinned down as can easily be done by direct visual
observation.
At first glance, it seems surprising that a cathode, which, as you can see in
any electron tube, glows perfectly evenly, does not necessarily emit electrons with
comparable uniformity. But the electron pattern usually reveals extensive patchiness
of varying intensities, from strongly emitting areas down to dark and dead spots
on the screen. The bright spots are said to have a lower "work function." This term
expresses in terms of electric charges and potentials, the force at the cathode
surface that resists ejection of thermally energized electrons. The electron emission
is subject to spectacular intensity variations because of the widely varying local
surface properties of the cathode material, which in turn results in local work-function
changes. Small differences in work-function values produce high contrasts in brightness,
since a physical law, very sensitive to the work-function term, establishes the
strength of the emission current.
What Is Being Done
As shown on the cover, a 21-inch screen displays such emission patterns. The
author, who in the picture is directing a photosensitive probe onto one crystal
plane of a multi-crystal emitter surface, conducts studies under a contract with
the Cambridge Research Laboratories of the US Air Force (Office of Aerospace Research,
Bedford, Mass.) on thin-film-coated nickel cathodes. Nickel, basically having a
high work function, would not lend itself to emission experiments in the rather
low temperature range of from 700 to 1,100°C, so an artificial activation process
has to be adopted. A thin barium film about 1,000 atomic layers thick is chemically
precipitated from an organic barium solution. It is then thermally evaporated down
to only a few atomic layers, until the crystal structure of the nickel gradually
comes into prominence on the phosphor screen. The thin barium coating lowers the
work function of the bare nickel surface.
As Fig. 2 reveals, very sharp grain structures become visible. Brightness is
uniform within their boundaries, indicating the consistency of uniform work-function
properties within each individual crystal face. It is due to the differences in
the packing density of nickel atoms in different crystal planes that the work function
varies.
Very interesting new phenomena were discovered with this potential tool in modern
cathode research. Among other effects, the so-called "emission reversal" was brought
into prominence. It consists of the appearance of higher emission currents at lower
temperatures. This seems to contradict the law of emission. The bright crystal face
near the picture center in Fig. 2 exhibits this phenomenon in a convincing manner.
It was taken at 890°C, while in the picture of the same cathode at 935°C
(Fig. 3), the brightness on the same crystal face is much lower.
With the help of the large-screen microscope we were able to discover that effects
like this were a direct result of barium droplet formations that cover certain faces
like a mesh-work and alter the straightforward concepts of uniform layer coverage.
Almost as exciting as a good love story to young people is visualizing evaporation
to the physicist. It is simply fascinating to watch on the large screen how barium
pools, forming on nickel with their typical "black eye" as depicted in Fig. 4, go
into a most spectacular vaporization act when heated to 1,100°C. They elongate and
develop a fuzzy tail structure just like a comet. The tail breaks up into round
globules that constantly sweep in the direction of the higher temperature region,
t2, gradually reducing in volume till they reach atomic size and disappear.
The emission microscope cannot, of course, follow the entire process down to the
atomic scale, but limits the observability to its own resolving power. The smallest
visible barium globule is 200 millimicrons in diameter.
Other important investigations now become feasible, such as those determining
the migration paths of active materials on hot surfaces and in studying the mechanism
of structural transformations to the point where the substances are completely dissipated
in vacuum.
Another successful application of this new instrument is for demonstrating the
emission mechanism of the modern impregnated tungsten matrix cathodes. These, also
known as impregnated dispenser cathodes, can draw high currents for thousands of
hours. The inventors of this important type of cathode knew its operational characteristics
for some time, by empirical measurements. However, their knowledge was confined
to critical points only, without knowing the actual physical phenomena connected
with them.
Again, the large screen of this instrument dissipated the fog that surrounded
the physical secrets of this type of cathode. What made this emitter tick suddenly
became clear. An initial state of pseudo-emission is shown in Fig. 5. Residuals
of the impregnant left on the surface after fabrication took the place of true dispenser
action of active barium. This state is succeeded by a profuse diffusion of liquid
barium through the pores of the sintered tungsten pellet, as visualized in Fig.
6.
Finally, at 1,230°C, a complete surface flooding indicates the end of the
desired activation, as seen in Fig. 7. (The windmill-like figure was etched into
the cathode to aid microscope centering.) Emission patchiness is a very undesirable
feature since, not only does the tube characteristic change, but load conditions
on the surface become non-uniform and noisiness in signal amplification increases.
These different stages of activation are a function of temperature, leading to
chemical conversions in the impregnant. They start at 400°C and are followed by
secondary conversions. After that, operation at 1,140°C guarantees during the tube
life an adequate continuous replenishment of active barium out of the pores by surface
migration along the tungsten.
These few examples may show that this new design concept in emission microscopy
is in a position to expand the stagnant field of cathode research. It also lends
itself to investigations on cold electron and ion emitters and may also play an
important role in metallurgical research.
* General Telephone & Electronics Laboratories Inc., Bayside 60, N.Y.
|