Module 18 - Radar PrinciplesPages
i,
1-1, 1-11,
1-21,
1-31,
1-41,
2-1,
2-11,
2-21,
2-31,
2-41,
3-1,
3-11,
3-21,
4-1,
4-11,
4-21,
AI-1,
AII-1,
Index-1 to 3
The number of
strikes per antenna revolution is referred to as HITS PER SCAN. During each revolution enough pulses must be
transmitted to return a usable echo. Note: The more pulses transmitted to a given area (at
slower antenna speeds), the greater the number of hits per scan. As an example, if the antenna rotates at 20
rPM, it completes a revolution in 3 seconds. During this time, a transmitter with a PRF of 200 pulses per second
(pps) transmits 600 pulses. Since 360 degrees of azimuth must be covered, the following formula shows the number
of pulses for each degree of azimuth:

Such a low number of pulses for any given target area greatly increases the likelihood that some targets will
be missed entirely; therefore, PRF and antenna speed must be matched for maximum efficiency. Q6.
Atmospheric interference with the travel of electromagnetic energy increases with what RF energy characteristic?
Q7. How is prt related to prf? Q8. What type of radar transmitter power is measured over a period of
time? Q9. What term is used to describe the product of pulse width and pulse-repetition frequency?
BEARING The TRUE BEARING (referenced to true north) of a radar target is the angle
between true north and a line pointed directly at the target. This angle is measured in the horizontal plane and
in a clockwise direction from true north. The bearing angle to the radar target may also be measured in a
clockwise direction from the centerline of your own ship or aircraft and is referred to as the RELATIVE BEARING.
Both true and relative bearing angles are illustrated in figure 1-8.

Figure 1-8. - True and relative bearings.
The antennas of most radar systems are designed to radiate energy in a one-directional lobe or beam
that can be moved in bearing simply by moving the antenna. As you can see in figure 1-9, the shape of the beam is
such that the echo signal strength varies in amplitude as the antenna beam moves across the target. At antenna
position A, the echo is minimal; at position B, where the beam axis is pointing directly at the target, the echo
strength is maximum. Thus, the bearing angle of the target can be obtained by moving the antenna to the
position at which the echo is strongest. In actual practice, search radar antennas move continuously; the point of
maximum echo return is determined by the detection circuitry as the beam passes the target or visually by the
operator. Weapons-control and guidance radar systems are positioned to the point of maximum signal return and
maintained at that position either manually or by automatic tracking circuits.

Figure 1-9. - Determination of bearing.
ALTITUDE Many radar systems are designed to determine only the range and bearing of an
object. Such radar systems are called TWO-DIMENSIONAL (2D) radars. In most cases these systems are further
described as SEARCH Radar Systems and function as early-warning devices that search a fixed volume of space. The
range and bearing coordinates provide enough information to place the target in a general area with respect to the
radar site and to determine distance, direction of travel, and relative speed. However, when action must be taken
against an airborne target, altitude must be known as well. a search radar system that detects altitude as well as
range and bearing is called a THREE-DIMENSIONAL (3D) radar. Altitude- or height-finding search radars use a
beam that is very narrow in the vertical plane. The beam is scanned in elevation, either mechanically or
electronically, to pinpoint targets. Height-finding radar systems that also determine bearing must have a beam
that is very narrow in both the vertical and horizontal planes. An electronic elevation-scanning pattern for a
search radar set is illustrated in figure 1-10. Lines originating at the antenna indicate the number of beam
positions required for complete elevation coverage. In practice the beams overlap slightly to prevent any gaps in
the coverage. Each beam position corresponds to a slight change in either the frequency or phase of the radiated
energy. a change in either phase or frequency of the energy causes it to leave the antenna at a different angle.
Thus, the frequency or
phase can be predetermined to create an orderly scanning pattern that covers the entire vertical
plane. Electronic scanning permits automatic compensation for an unstable radar platform (site), such as a ship at
sea. Error signals are produced by the roll and pitch of the ship and are used to correct the radar beam to ensure
complete elevation coverage.

Figure 1-10. - Electronic elevation scan.
Mechanical elevation scanning is achieved by mechanically moving the antenna or radiation source.
Weapons-control and tracking radar systems commonly use mechanical elevation scanning techniques. Most
electronically scanned radar systems are used as air search radars. Some older air-search radar systems use a
mechanical elevation scanning device; however, these are being replaced by electronically scanned radar systems.
Q10. What type of target bearing is referenced to your ship? Q11. What type of radar detects
range, bearing, and height? Q12. What characteristic(s) of radiated energy is (are) altered to achieve electronic scanning? TARGET RESOLUTION The TARGET RESOLUTION of a radar is its ability to distinguish between
targets that are very close together in either range or bearing. Weapons-control radar, which requires great
precision, should be able to distinguish between targets that are only yards apart. Search radar is usually less
precise and only distinguishes between targets that are hundreds of yards or even miles apart. Resolution is
usually divided into two categories; RANGE RESOLUTION and BEARING RESOLUTION. Range Resolution
Range resolution is the ability of a radar system to distinguish between two or more targets on the same bearing
but at different ranges. The degree of range resolution depends on the width of the transmitted pulse, the types
and sizes of targets, and the efficiency of the receiver and indicator. Pulse width is the primary factor in range
resolution. a well-designed radar system, with all other factors at maximum efficiency, should be able to
distinguish targets separated by one-half the pulse width time. Therefore, the theoretical range resolution of a
radar system can be calculated from the following formula:

The above formula is often written as:

For example, if a radar system has a pulse width of 5 microseconds, the range resolution is calculated as
follows:

In the above example, targets on the same bearing would have to be separated by more than 820 yards to show up
as two targets on your indicator. Bearing Resolution Bearing, or azimuth,
resolution is the ability of a radar system to separate objects at the same range but at different bearings. The
degree of bearing resolution depends on radar beam width and the range of the targets. Range is a factor in
bearing resolution because the radar beam spreads out as range increases. a Radar BEAM is defined in width in
terms of Half-Power PointS. All the points off the centerline of the beam that are at one-half the power level at
the center are plotted to define beam width. When the half-power points are connected to the antenna by a curve,
such as that shown in figure 1-11, the resulting angular width of the curve is called the Antenna BEAM WIDTH. The
physical size and shape of the antenna determines beam width. Beam width can vary from about 1 degree up to 60
degrees. In figure 1-11, only the target within the half-power points will reflect a useful echo. Two targets at
the same range must be separated by at least one beam width to be distinguished as two objects.

Figure 1-11. - Beam half-power points.
Radar ACCURACY Radar accuracy is a measure of the ability of a radar system to determine the correct range, bearing, and, in
some cases, height of an object. The degree of accuracy is primarily determined by the resolution of the radar
system. Some additional factors affecting accuracy are pulse shape and atmospheric conditions.
Pulse Shape In the case of a pulse radar, the shape and width of the RF pulse influences minimum
range, range accuracy, and maximum range. The ideal pulse shape is a square wave having vertical leading and
trailing edges. However, equipments do not usually produce the ideal waveforms. The factors influencing
minimum range are discussed first. Since the receiver cannot receive target reflections while the transmitter is
operating, you should be able to see that a narrow pulse is necessary for short ranges. a sloping trailing edge
extends the width of the transmitter pulse, although it may add very little to the total power generated.
Therefore, along with a narrow pulse, the trailing edge should be as near vertical as possible. A sloping
leading edge also affects minimum range as well as range accuracy since it provides no definite point from which
to measure elapsed time on the indicator time base. Using a starting point at the lower edge of the pulse's
leading edge would increase minimum range. Using a starting point high up on the slope would reduce the accuracy
of range measurements at short ranges which are so vital for accurate solution of the fire-control problem.
Maximum range is influenced by pulse width and pulse repetition frequency (prf). Since a target can reflect only a
very small part of the transmitted power, the greater the transmitted power, the greater the strength of the echo
that could be received. Thus, a transmitted pulse should quickly rise to its maximum amplitude, remain at this
amplitude for the duration of the desired pulse width, and decay instantaneously to zero. Figure 1-12 illustrates
the effects of pulse shapes.

Figure 1-12. - Pulse shapes and effects.
Atmospheric Conditions Electromagnetic wavefronts travel through empty space in straight
lines at the speed of light, but the REFRACTIVE Index of the atmosphere affects both the travel path and the
speed of the
electromagnetic wavefront. The path followed by electromagnetic energy in the atmosphere, whether
direct or reflected, usually is slightly curved; and the speed is affected by temperature, atmospheric pressure,
and the amount of water vapor present in the atmosphere, which all affect the refractive index. As altitude
increases, the combined effects of these influences, under normal atmospheric conditions, cause a small, uniform
increase in signal speed. This increase in speed causes the travel path to curve slightly downward, as shown in
figure 1-13. The downward curve extends the radar horizon beyond a line tangent to the earth, as illustrated in
figure 1-14.
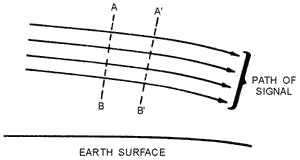
Figure 1-13. - Wavefront path.

Figure 1-14. - Extension of the radar horizon. The reason for the downward curve can be illustrated using line AB in figure 1-13. Line AB represents
the surface of a wavefront with point a higher in altitude than point B. As wavefront AB moves to the point
represented by A'B', the speed at a and A'is faster than the speed at B and B'since a and A'are at a greater
altitude. Therefore, in a given time, the upper part of the wavefront moves farther than the lower part. The
wavefront leans slightly forward as it moves. Since the direction of energy propagation is always perpendicular to
the surface of a wavefront, the tilted wavefront causes the energy path to curve downward.
REFRACTION is the bending of electromagnetic waves caused by a change in the density of the medium
through which the waves are passing. a visible example of electromagnetic refraction is the apparent displacement
of underwater objects caused by the bending of light as it passes from the atmosphere into the water. An Index of
REFRACTION has been established which indicates the degree of refraction, or bending, caused by different
substances. Because the density of the atmosphere changes with altitude, the index of refraction changes gradually
with height. The temperature and moisture content of the atmosphere normally decrease uniformly with an
increase in altitude. However, under certain conditions the temperature may first increase with height and then
begin to decrease. Such a situation is called a temperature inversion. An even more important deviation from
normal may exist over the ocean. Since the atmosphere close to the surface over large bodies of water may contain
more than a normal amount of moisture, the moisture content may decrease more rapidly at heights just above the
sea. This effect is referred to as MOIsTURE LAPSE. Either temperature inversion or moisture lapse, alone
or in combination, can cause a large change in the refraction index of the lowest few-hundred feet of the
atmosphere. The result is a greater bending of the radar waves passing through the abnormal condition. The
increased bending in such a situation is referred to as DUCTING and may greatly affect radar performance. The
radar horizon may be extended or reduced, depending on the direction the radar waves are bent. The effect of
ducting on radar waves is illustrated in figure 1-15. 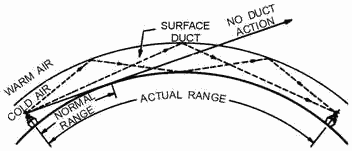
Figure 1-15. - Ducting effect on the radar wave. Another effect of the atmosphere on radar performance is caused by particles suspended in the air. Water
droplets and dust particles diffuse radar energy through absorption, reflection, and scattering so less energy
strikes the target. Consequently, the return echo is smaller. The overall effect is a reduction in usable range
that varies widely with weather conditions. The higher the frequency of a radar system, the more it is affected by
weather conditions such as rain or clouds. In some parts of the world, dust suspended in the air can greatly
decrease the normal range of high-frequency radar. Q13. What term is used to describe the ability of a
radar system to distinguish between targets that are close together? Q14. The degree of bearing resolution
for a given radar system depends on what two factors? Q15. What happens to the speed of electromagnetic
energy traveling through air as the altitude increases?
Q16. What term is used to describe a situation in which atmospheric temperature first increases with
altitude and then begins to decrease? Radar Principles of Operation Radar systems, like other complex electronics systems, are composed of several major subsystems and many
individual circuits. This section will introduce you to the major subsystems common to most radar sets. a brief
functional description of subsystem principles of operation will be provided. a much more detailed explanation of
radar subsystems will be given in chapters 2 and 3. Since most radar systems in use today are some variation of
the pulse radar system, the units discussed in this section will be those used in pulse radar. All other types of
radar use some variation of these units, and these variations will be explained as necessary.
Radar COMPONENTS
Pulse radar systems can be functionally divided into the six essential components shown in figure 1-16.
These components are briefly described in the following paragraphs and will be explained in detail after that:

Figure 1-16. - Functional block diagram of a basic radar system. • The Synchronizer (also referred to as the TIMER or KEYER) supplies the synchronizing signals that time
the transmitted pulses, the indicator, and other associated circuits. • The Transmitter generates
electromagnetic energy in the form of short, powerful pulses. • The DUPLEXER allows the same antenna to be used for transmitting and receiving. • The Antenna System routes the electromagnetic energy from the
transmitter, radiates it in a highly directional beam, receives any returning echoes, and routes those echoes to
the receiver. • The RECEIVER amplifies the weak, electromagnetic pulses returned from the reflecting object
and reproduces them as video pulses that are sent to the indicator.
• The INDICATOR produces a visual indication of the echo pulses in a manner that, at a minimum,
furnishes range and bearing information. While the physical configurations of radar systems differ, any radar
system can be represented by the functional block diagram in figure 1-16. An actual radar set may have several of
these functional components within one physical unit, or a single one of these functions may require several
physical units. However, the functional block diagram of a basic radar set may be used to analyze the operation
of almost any radar set.
In the following paragraphs, a brief description of the operation of each of the major components is given.
Synchronizer (Timer) The synchronizer ensures that all circuits connected with the
radar system operate in a definite timed relationship. It also times the interval between transmitted pulses to
ensure that the interval is of the proper length. Timing pulses are used to ensure synchronous circuit operation
and are related to the prf. The PRF can be set by any stable oscillator, such as a sine-wave oscillator,
multivibrator, or a blocking oscillator. That output is then applied to pulse-shaping circuits to produce timing
pulses. Associated components can be timed by the output of the synchronizer or by a timing signal from the
transmitter as it is turned on. Transmitter The transmitter generates powerful
pulses of electromagnetic energy at precise intervals. The required power is obtained by using a high-power
microwave oscillator, such as a magnetron, or a microwave amplifier, such as a klystron, that is supplied by a
low-power RF source. (The construction and operation of microwave components can be reviewed in NEETS, Module 11,
Microwave Principles.) The high-power generator, whether an oscillator or amplifier, requires operating power in
the form of a properly-timed, high-amplitude, rectangular pulse. This pulse is supplied by a transmitter unit
called the Modulator. When a high-power oscillator is used, the modulator high-voltage pulse switches the
oscillator on and off to supply high-power electromagnetic energy. When a microwave power amplifier is used, the
modulator pulse activates the amplifier just before the arrival of an electromagnetic pulse from a preceding stage
or a frequency-generation source. Normally, because of the extremely high voltage involved, the modulator pulse is
supplied to the cathode of the power tube and the plate is at ground potential to shield personnel from shock
hazards. The modulator pulse may be more than 100,000 volts in high-power radar transmitters. In any case, radar
transmitters produce voltages, currents, and radiation hazards that are extremely dangerous to personnel. Safety
precautions must always be strictly observed when working in or around a radar transmitter.
Duplexer A duplexer is essentially an electronic switch that permits a radar system to use a
single antenna to both transmit and receive. The duplexer must connect the antenna to the transmitter and
disconnect the antenna from the receiver for the duration of the transmitted pulse. The receiver must be
completely isolated from the transmitted pulse to avoid damage to the extremely sensitive receiver input
circuitry. After the transmitter pulse has ended, the duplexer must rapidly disconnect the transmitter and connect
the receiver to the antenna. As previously mentioned, the switching time is called receiver recovery time, and
must be very fast if close-in targets are to be detected. Additionally, the duplexer should absorb very little
power during either phase of operation. Low-loss characteristics are particularly important during the receive
period of duplexer operation. This is because the received signals are of extremely low amplitude.
Antenna System The antenna system routes the pulse from the transmitter, radiates
it in a directional beam, picks up the returning echo, and passes it to the receiver with a minimum of loss. The
antenna system includes the antenna, transmission lines and waveguide from the transmitter to the antenna, and the
transmission line and waveguide from the antenna to the receiver. In some publications the duplexer is included as
a component of the antenna system. Receiver The receiver accepts the weak echo
signals from the antenna system, amplifies them, detects the pulse envelope, amplifies the pulses, and then routes
them to the indicator. One of the primary functions of the radar receiver is to convert the frequency of the
received echo signal to a lower frequency that is easier to amplify. This is because radar frequencies are very
high and difficult to amplify. This lower frequency is called the INTERMEDIATE Frequency (IF). The type of
receiver that uses this frequency conversion technique is the SUPER HETERODYNE RECEIVER. Superheterodyne receivers used in radar systems must have good stability and extreme sensitivity. Stability is ensured by careful design and
the overall sensitivity is greatly increased by the use of many IF stages. Indicator
The indicator uses the received signals routed from the radar receiver to produce a visual indication of target
information. The cathode-ray oscilloscope is an ideal instrument for the presentation of radar data. This is
because it not only shows a variation of a single quantity, such as voltage, but also gives an indication of the
relative values of two or more quantities. The sweep frequency of the radar indicator is determined by the
pulse-repetition frequency of the radar system. Sweep duration is determined by the setting of the range-selector
switch. Since the indicator is so similar to an oscilloscope, the term Radar SCOPE is commonly used when referring
to radar indicators. Q17. What radar subsystem supplies timing signals to coordinate the operation of
the complete system? Q18. When a transmitter uses a high-power oscillator to produce the output pulse,
what switches the oscillator on and off? Q19. What radar component permits the use of a single antenna
for both transmitting and receiving? SCANNING
Radar systems are often identified by the type of SCANNING the system uses. Scanning is the systematic
movement of a radar beam in a definite pattern while searching for or tracking a target. The type and method of
scanning used depends on the purpose and type of radar and on the antenna size and design. In some cases, the type
of scan will change with the particular system mode of operation. For example, in a particular radar system, the
search mode scan may be quite different from that of the track mode scan. Stationary-Lobe
Scanning
A Single STATIONARY-LOBE SCANNING System is the simplest type of scanning. This method produces a single beam
that is stationary in relation to the antenna. The antenna is then mechanically rotated continuously to obtain
complete 360-degree azimuth coverage. a stationary lobe, however, cannot satisfactorily track a moving object
because it does not provide enough information about the object's movement to operate automatic tracking circuits,
such as those in fire-control tracking
- |
Matter, Energy,
and Direct Current |
- |
Alternating Current and Transformers |
- |
Circuit Protection, Control, and Measurement |
- |
Electrical Conductors, Wiring Techniques,
and Schematic Reading |
- |
Generators and Motors |
- |
Electronic Emission, Tubes, and Power Supplies |
- |
Solid-State Devices and Power Supplies |
- |
Amplifiers |
- |
Wave-Generation and Wave-Shaping Circuits |
- |
Wave Propagation, Transmission Lines, and
Antennas |
- |
Microwave Principles |
- |
Modulation Principles |
- |
Introduction to Number Systems and Logic Circuits |
- |
- Introduction to Microelectronics |
- |
Principles of Synchros, Servos, and Gyros |
- |
Introduction to Test Equipment |
- |
Radio-Frequency Communications Principles |
- |
Radar Principles |
- |
The Technician's Handbook, Master Glossary |
- |
Test Methods and Practices |
- |
Introduction to Digital Computers |
- |
Magnetic Recording |
- |
Introduction to Fiber Optics |
Note: Navy Electricity and Electronics Training
Series (NEETS) content is U.S. Navy property in the public domain. |
|